Introduction
Conventional/historical vaccine design
Classically, vaccine design has been predicated on what is termed forward vaccinology, where surrogates of natural infection (e.g., killed pathogens, attenuated strains, toxoids, or protein subunits), have generated highly successful vaccines. Indeed, scourges, such as smallpox, polio, and measles that plagued humanity for millennia either have been rendered exceedingly uncommon or have, by-in-large, been eradicated and swept into the dustbin of history. Although cell-mediated responses (Th1) are almost definitely a component of traditional vaccines, the forward vaccinology gold standard correlate of vaccine protective efficacy is the generation of functional antibodies.
Need for new modalities
Success with traditional design strategies notwithstanding, the list of FDA-approved vaccines against human viruses is surprisingly short. Nontraditional, new technological approaches, such as mRNA-based strategies and reverse vaccinology, promise to augment the vaccine arsenal (Sharma S et al, 2019; Jackson NAC et al, 2020; Reichmuth AM et al, 2016).
COVID-19 and the rise of mRNA vaccines
Although the concept of mRNA vaccines dates back to the previous century (Ying H et al, 1999), 2020 saw mRNA vaccines abruptly thrust into the limelight because of the emergence of COVID-19, severe acute respiratory syndrome coronavirus 2 (SARS-CoV-2).
mRNA vaccines that have been designed to address COVID-19
Two mRNA vaccines, COMIRNATY® (BNT162b2), which is made by Pfizer and BioNTech, and Spikevax® (mRNA-1273), which is made by Moderna, have had their BLAs approved by the United States Food and Drug Administration but are still being manufactured and administered under Emergency Use Authorization status.
Immune responses generated by COVID-19 mRNA vaccines
Innate immune responses
The first step toward immunogenicity begins with the innate immune system. After injection and uptake by the myocyte, it is postulated that the antigen is transferred via a cross priming mechanism to bone marrow-derived antigen presenting cells that then induce MHC class-I restricted CD8+ T cells (Lazzaro S et al, 2015) in the periphery and in germinal centers (GCs). A certain portion of the vaccine must directly find its way to the lymph nodes as GCs contain vaccine mRNA and spike antigen up to 8 weeks postvaccination (Röltgen K et al, 2022).
The BNT162b2 SARS-CoV-2 mRNA vaccine modulates both monocytes and natural killer (NK) cells, the two main populations that are responsible for innate immune responses to pathogens. It has been shown that vaccination significantly increased: 1) classical and intermediate inflammatory monocytes, 2) CD56bright, CD56dim, and CD56dim/CD16dim NK cells, and 3) IFN-γ+ production as well as perforin and granzyme content by NK cells (Saresella M et al, 2022).
The innate immune response in response to vaccination likely is very different than that which occurs during an actual COVID-19 infection for the following reasons:
- Positron-emission tomography scans of recently mRNA vaccinated people showed what was described as an increased acute inflammatory reaction in deltoid muscle followed by immune reaction in the lymph nodes linked to humoral immunity (Kubota K et al, 2022).
- Viral single-stranded mRNA is inherently immunogenic as it is a pathogen-associated molecular pattern molecule that is detected by endosomal Toll-like receptors TLR7 and TLR8 (Zhang Z et al, 2016; Tanji H et al, 2015). However, modified RNAs have nonatural bases, such pseudouridine (Karikó K et al, 2008; Carlile TM et al, 2014) and 1-methylpseudouridine (Andries O et al, 2014) that facilitate translation but attenuate immunogenicity (Karikó K et al, 2011; Karikó K et al, 2008; Kariko K et al, 2008) and prevent the activation of TLR7, TLR8 and other innate immune sensors (Anderson BR et al, 2010; Anderson BR et al, 2011).
- COVID-19 mRNA vaccines disrupt and do not elicit immune responses through Type I interferon signaling, which has been demonstrated to be very important to overcoming COVID-19 infections (Seneff S et al, 2022).
Adaptive immune responses
T-cell -generated responses mRNA vaccines
COVID-19 mRNA vaccines have been shown to be capable of producing antigen protein along with concomitant innate immune responses that prime both CD8+ and CD4+ T-cells to differentiate into effector and memory subsets (Teijaro JR et al, 2021). Moreover, circulating spike protein-specific follicular helper T-cells (TFH) cells, which represent a specialized subset of CD4+ T cells that provide critical signals for B cell maturation (Pardi N et al, 2018), correlate with neutralizing antibodies and increase during early convalescence (Boppana S et al, 2021). TFH cells peak one week after the second immunization and are maintained for at least another six months (Mudd PA .et al, 2022); it remains to be deciphered how long spike protein-specific TFH cells persist after vaccination.
Antibody-generated responses to mRNA vaccines
The importance of sustained humoral immunity is widely recognized, and with regard to established vaccines, humoral antiviral responses are extraordinarily stable (Amanna IJ et al, 2007) as many vaccines exhibit only a 5% to 10% yearly decrease in the neutralizing antibody levels (Davidkin I et al, 2008; Seagle EE et al, 2018). However, as shown in Table 1, the humoral responses generated in response to the COVID mRNA vaccination have not reached this level of durability.
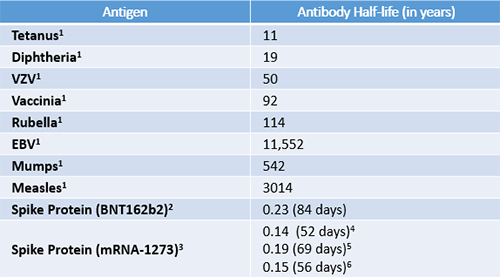
Duration of antibody responses elicited by COVID-19 mRNA vaccines compared to established vaccines. EBV = Epstein-Barr virus; VZV = Varicella zoster virus. 1. Values were obtained from Amanna IJ et al, 2007. 2. Values were obtained from Shrotri M et al, 2021. 3. Values were obtained from Doria-Rose N et al, 2021 using an exponential decay model. 4. All binding antibodies. 5. Pseudovirus neutralization. 6. Live virus neutralization
The humoral response in response to mRNA vaccination likely is considerably different than that which occurs during an actual COVID-19 infection for the following reasons:
- In recovered individuals, both IgG and neutralizing antibody levels decrease only modestly at 8 to 10 months after the infection (Dan JM et al, 2021; Vanshylla K et al, 2021). In contrast, vaccine plasma and saliva spike and RBD-specific IgG concentrations decrease from their peak values by approximately 20-fold by 9 months after primary vaccination (Röltgen K et al, 2022).
- There is substantially lower incidence of breakthrough infection among previously infected persons than among vaccinated persons (Anderson M et al 2021; Hall VJ et al, 2021).
- Vaccination is heavily biased toward IgG responses, whereas infection is biased toward IgMs and IgAs. Antibody breadth against viral variants is lower after infection than mRNA vaccines but improves over several months (Röltgen K et al, 2022) and indicates ongoing clonal evolution (Wang Z et al 2021).
- Vaccines have not been demonstrated to generate long-lived plasma cells in a SARS-CoV-2 virus naïve population (Giannotta G. et al, 2021). In contrast, SARS-CoV-2 infection produces a memory compartment consistent with long-term persistence of germinal centers 12 months post infection (Wang Z et al 2021).
- SARS-CoV-2 protein and nucleic acids have been reported to remain in the gut for at least two months after infection (Gaebler C et al, 2021); to date, there is no evidence that COVID vaccine mRNAs or proteins persist in this tissue.
- SARS-CoV-2 spike-specific antibodies induce natural killer cell-mediated antibody-dependent cellular cytotoxicity (ADCC) in patients with natural infection and vaccinated persons. Although found at lower levels, antibodies elicited by natural infection induced a significantly stronger ADCC response compared to those induced by vaccination (Rieke GJ et al, 2022).
IgA — The forgotten immunoglobulin
IgA is the most abundantly produced Ig in humans (66 gm/kg/day) and the most abundant isotype at mucosal sites (Underdown BJ et al, 1998; Vidarsson G et al, 2014). Although not currently a correlate of vaccine protection, IgA production, both in serum and at the mucosa, should be for the following reasons:
- IgA possesses superior anti-viral properties vs. IgG for influenza and for SARS-CoV-2 (Muramatsu et al, 2014; Liew FY et al, 2014; Wang Z et al, 2021; Sterlin D et al, 2021; Chao YX et al, 2020).
- Serum IgA is 7-fold more potent than serum IgG in viral neutralization; temporal changes in circulating IgA+ plasmablasts with mucosal homing potential were detected during infection along with neutralizing IgA in airway fluid and saliva (Sterlin D et al, 2021).
-Although SARS-CoV-2 spike-specific serum IgA levels decline quickly after infection, local concentrations at mucosal surfaces persist longer and include dimeric isoforms with potent neutralizing capacity, which are 15X greater than monomeric IgA (Wang Z et al, 2021; Sterlin D et al, 2021). - IgA antibodies have been demonstrated to afford protection from a coronavirus, avian infectious bronchitis (Toro H et al, 1994).
Next generation COVID-19 mRNA vaccines—Back to the future
Limitations of the current COVID-19 mRNA vaccines
COVID-19 mRNA vaccines offered great hope of ending the pandemic as preliminary studies indicated that BNT162b2 was 94% to 95% effective in preventing symptomatic coronavirus COVID-19 7 days or more after receipt of the second dose of vaccine (Polack FP et al, 2020; Dagan N et al, 2021; Haas EJ et al, 2021; Chodick G et al, 2022) and that mRNA-1273 was 94% effective in preventing COVID-19 (Baden LR et al, 2021). Initial promise notwithstanding, as we approach 2023, COVID outbreaks and reinfections are still occurring, new variants are a constant cause of concern, and questions are rapidly accumulating about overall efficacy and potential unanticipated and unaddressed safety issues.
Next generation COVID-19 mRNA vaccines—Following the science
The following features are needed for the development of next-generation vaccines that can address the current set of challenges:
- Application of reverse vaccinology approaches, the central tenet of which is first identifying antigenic proteins and then finding immunodominant B-cell epitopes.
- Prolonged host immunity that both prevents individuals from being infected and from infecting others.
- More accurate recapitulation of an actual infection.
-mRNA in the form of a self-amplifying construct delivered in a virus-like particle (Biddlecome A et al, 2019). - True multivalent vaccines that incorporate other COVID-19 proteins, such as the N and M proteins, in addition to the Spike protein.
-Mitigation against the evolution of new variants that give rise to vaccine resistance as a result of monovalency (Kennedy DA et al, 2018).
-N proteins of many coronaviruses are highly immunogenic, expressed abundantly during infection (Cong Y et al, 2020), and accumulated fewer mutations over time than other coronavirus proteins (Grifoni A et al, 2020).
-N antibodies neutralize SARS-CoV-2 intracellularly (Albecka A et al, 2021). - Bias toward IgA and mucosal immunity.
- Assurance that the mRNA used is not immunogenic (does not generate anti-drug antibodies) and does not generate antibodies that disrupt extracellular RNA and its functionality (Kluever AK et al, 2019).
- Assurance that the antibodies generated are not anti-idiotypic (Murphy WJ et al, 2022).
- Design strategies to minimize GC content and G-quadraplex structures and eliminate nonatural bases in the mRNA (Seneff S et al, 2022).
References
Albecka A, Clift D, Vaysburd M, Rhinesmith T, Caddy SL, Favara DM, Baxendale HE, James LC. A functional assay for serum detection of antibodies against SARS-CoV-2 nucleoprotein. EMBO J. 2021 Sep 1;40(17):e108588.
Amanna IJ, Slifka MK, Crotty S. Immunity and immunological memory following smallpox vaccination. Immunol Rev. 2006 Jun;211:320-37.
Amanna IJ, Carlson NE, Slifka MK. Duration of humoral immunity to common viral and vaccine antigens. N Engl J Med. 2007 Nov 8;357(19):1903-15.
Andries O, et al. N1-methylpseudouridine-incorporated mRNA outperforms pseudouridine-incorporated mRNA by providing enhanced protein expression and reduced immunogenicity in mammalian cell lines and mice. J Control Release. 2015; 217:337–344.
Anderson M, Stec M, Rewane A, Landay A, Cloherty G, Moy J. SARS-CoV-2 antibody responses in infection-naïve or previously infected individuals after 1 and 2 doses of the BNT162b2 vaccine. JAMA Netw Open 2021.
Anderson BR, et al. Incorporation of pseudouridine into mRNA enhances translation by diminishing PKR activation. Nucleic Acids Res. 2010; 38:5884–5892.
Anderson BR, et al. Nucleoside modifications in RNA limit activation of 2′–5′-oligoadenylate synthetase and increase resistance to cleavage by RNase L. Nucleic Acids Res. 2011; 39:9329–9338.
Baden LR, El Sahly HM, Essink B, et al. Efficacy and safety of the mRNA-1273 SARS-CoV-2 vaccine. N Engl J Med 2021;384:403-416.
Biddlecome A, Habte HH, McGrath KM, Sambanthamoorthy S, Wurm M, Sykora MM, Knobler CM, Lorenz IC, Lasaro M, Elbers K, Gelbart WM. Delivery of self-amplifying RNA vaccines in in vitro reconstituted virus-like particles. PLoS One. 2019 Jun 4;14(6):e0215031.
Carlile TM, et al. Pseudouridine profiling reveals regulated mRNA pseudouridylation in yeast and human cells. Nature. 2014; 515:143–146
Chao YX, Rötzschke O, Tan EK. The role of IgA in COVID-19. Brain Behav Immun. 2020 Jul;87:182-183.
Chodick G, Tene L, Rotem RS, Patalon T, Gazit S, Ben-Tov A, Weil C, Goldshtein I, Twig G, Cohen D, Muhsen K, The Effectiveness of the Two-Dose BNT162b2 Vaccine: Analysis of Real-World Data, Clinical Infectious Diseases, Volume 74, Issue 3, 1 February 2022, Pages 472–478
Cong Y, Ulasli M, Schepers H, et al. Nucleocapsid Protein Recruitment to Replication-Transcription Complexes Plays a Crucial Role in Coronaviral Life Cycle. J Virol. 2020 Jan 31;94(4):e01925-19.
Dagan N, Barda N, Kepten E, et al. BNT162b2 mRNA Covid-19 vaccine in a nationwide mass vaccination setting. N Engl J Med 2021;384:1412-1423.
Dan JM, Mateus J, Kato Y, et al. Immunological memory to SARS-CoV-2 assessed for up to 8 months after infection. Science 2021;371(6529)
Davidkin I, Jokinen S, Broman M, Leinikki P, Peltola H. Persistence of measles, mumps, and rubella antibodies in an MMR-vaccinated cohort: a 20-year follow-up. J Infect Dis 2008;197:950-956.
Doria-Rose N, Suthar MS, Makowski M, O'Connell S, McDermott AB, Flach B, Ledgerwood JE, Mascola JR, Graham BS, Lin BC, O'Dell S, Schmidt SD, Widge AT, Edara VV, Anderson EJ, Lai L, Floyd K, Rouphael NG, Zarnitsyna V, Roberts PC, Makhene M, Buchanan W, Luke CJ, Beigel JH, Jackson LA, Neuzil KM, Bennett H, Leav B, Albert J, Kunwar P; mRNA-1273 Study Group. Antibody Persistence through 6 Months after the Second Dose of mRNA-1273 Vaccine for Covid-19. N Engl J Med. 2021 Jun 10;384(23):2259-2261.
Gaebler C, et al. Evolution of antibody immunity to SARS-CoV-2. Nature. 2021;591:639–644.
Giannotta G, Giannotta N. mRNA COVID-19 Vaccines and Long-Lived Plasma Cells: A Complicated Relationship. Vaccines (Basel). 2021 Dec 20;9(12):1503.
Grifoni A, Weiskopf D, Ramirez SI, et al. Targets of T Cell Responses to SARS-CoV-2 Coronavirus in Humans with COVID-19 Disease and Unexposed Individuals. Cell. 2020 Jun 25;181(7):1489-1501.e15.
Haas EJ, Angulo FJ, McLaughlin JM, et al. Impact and effectiveness of mRNA BNT162b2 vaccine against SARS-CoV-2 infections and COVID-19 cases, hospitalisations, and deaths following a nationwide vaccination campaign in Israel: an observational study using national surveillance data. Lancet 2021;397:1819-1829.
Hall VJ, Foulkes S, Charlett A, et al. SARS-CoV-2 infection rates of antibody-positive compared with antibody-negative health-care workers in England: a large, multicentre, prospective cohort study (SIREN). Lancet 2021;397:1459-1469.
Jackson NAC, Kester KE, Casimiro D, et al. The promise of mRNA vaccines: a biotech and industrial perspective. NPJ Vaccines. 2020 Feb 4;5:11.
Kariko K, et al. Incorporation of pseudouridine into mRNA yields superior nonimmunogenic vector with increased translational capacity and biological stability. Mol Ther. 2008; 16:1833–1840.
Karikó K, Muramatsu H, Welsh FA, et al. Incorporation of pseudouridine into mRNA yields superior nonimmunogenic vector with increased translational capacity and biological stability. Mol Ther. 2008 Nov;16(11):1833-40.
Kariko K, Buckstein M, Ni H, Weissman D. Suppression of RNA recognition by Toll-like receptors: the impact of nucleoside modification and the evolutionary origin of RNA. Immunity. 2005; 23:165–175.
Karikó K, Muramatsu H, Ludwig J, et al. Generating the optimal mRNA for therapy: HPLC purification eliminates immune activation and improves translation of nucleoside-modified, protein-encoding mRNA. Nucleic Acids Res. 2011 Nov;39(21):e142.
Kennedy DA, Read AF. Why the evolution of vaccine resistance is less of a concern than the evolution of drug resistance. Proc Natl Acad Sci U S A. 2018 Dec 18;115(51):12878-12886. doi: 10.1073/pnas.1717159115.
Kluever AK, Braumandl A, Fischer S, Preissner KT, Deindl E. The Extraordinary Role of Extracellular RNA in Arteriogenesis, the Growth of Collateral Arteries. Int J Mol Sci. 2019 Dec 7;20(24):6177.
Kubota K, Saginoya T, Ishiwata K, Nakasato T, Munechika H. [18F]FDG uptake in axillary lymph nodes and deltoid muscle after COVID-19 mRNA vaccination: a cohort study to determine incidence and contributing factors using a multivariate analysis. Ann Nucl Med. 2022 Apr;36(4):340-350.
Lazzaro S, Giovani C, Mangiavacchi S, et al. CD8 T-cell priming upon mRNA vaccination is restricted to bone-marrow-derived antigen-presenting cells and may involve antigen transfer from myocytes. Immunology. 2015 Oct;146(2):312-26.
Liew FY, Russell SM, Appleyard G, Brand CM, Beale J. Cross-protection in mice infected with influenza A virus by the respiratory route is correlated with local IgA antibody rather than serum antibody or cytotoxic T cell reactivity. Eur J Immunol. 1984;14(4):350–6.
Mudd PA, Minervina AA, Pogorelyy MV, Turner JS, Kim W, Kalaidina E, Petersen J, Schmitz AJ, Lei T, Haile A, Kirk AM, Mettelman RC, Crawford JC, Nguyen THO, Rowntree LC, Rosati E, Richards KA, Sant AJ, Klebert MK, Suessen T, Middleton WD; SJTRC Study Team, Wolf J, Teefey SA, O'Halloran JA, Presti RM, Kedzierska K, Rossjohn J, Thomas PG, Ellebedy AH. SARS-CoV-2 mRNA vaccination elicits a robust and persistent T follicular helper cell response in humans. Cell. 2022 Feb 17;185(4):603-613.
Muramatsu M, Yoshida R, Yokoyama A, Miyamoto H, Kajihara M, Maruyama J, et al. Comparison of antiviral activity between IgA and IgG specific to influenza virus hemagglutinin: increased potential of IgA for heterosubtypic immunity. PLoS One. 2014;9(1):e85582.
Murphy WJ, Longo DL. A Possible Role for Anti-idiotype Antibodies in SARS-CoV-2 Infection and Vaccination. N Engl J Med. 2022 Jan 27;386(4):394-396.
Pardi N, Hogan MJ, Porter FW, Weissman D. mRNA vaccines - a new era in vaccinology. Nat Rev Drug Discov. 2018 Apr;17(4):261-279.
Polack FP, Thomas SJ, Kitchin N, et al. Safety and efficacy of the BNT162b2 mRNA Covid-19 vaccine. N Engl J Med 2020;383:2603-2615.
Reichmuth AM, Oberli MA, Jaklenec A, et al. mRNA vaccine delivery using lipid nanoparticles. Ther Deliv. 2016;7(5):319-34.
Rieke GJ, van Bremen K, Bischoff J, ToVinh M, Monin MB, Schlabe S, Raabe J, Kaiser KM, Finnemann C, Odainic A, Kudaliyanage A, Latz E, Strassburg CP, Boesecke C, Schmidt SV, Krämer B, Rockstroh JK, Nattermann J. Natural Killer Cell-Mediated Antibody-Dependent
Röltgen K, Nielsen SCA, Silva O, Younes SF, Zaslavsky M, Costales C, Yang F, Wirz OF, Solis D, Hoh RA, Wang A, Arunachalam PS, Colburg D, Zhao S, Haraguchi E, Lee AS, Shah MM, Manohar M, Chang I, Gao F, Mallajosyula V, Li C, Liu J, Shoura MJ, Sindher SB, Parsons E, Dashdorj NJ, Dashdorj ND, Monroe R, Serrano GE, Beach TG, Chinthrajah RS, Charville GW, Wilbur JL, Wohlstadter JN, Davis MM, Pulendran B, Troxell ML, Sigal GB, Natkunam Y, Pinsky BA, Nadeau KC, Boyd SD. Immune imprinting, breadth of variant recognition, and germinal center response in human SARS-CoV-2 infection and vaccination. Cell. 2022 Mar 17;185(6):1025-1040.e14.
Saresella M, Piancone F, Marventano I, Hernis A, Trabattoni D, Invernizzi M, La Rosa F, Clerici M. Innate immune responses to three doses of the BNT162b2 mRNA SARS-CoV-2 vaccine. Front Immunol. 2022 Aug 22;13:947320.
Seagle EE, Bednarczyk RA, Hill T, et al. Measles, mumps, and rubella antibody patterns of persistence and rate of decline following the second dose of the MMR vaccine. Vaccine 2018;36:818-826.
Seneff S, Nigh G, Kyriakopoulos AM, McCullough PA. Innate immune suppression by SARS-CoV-2 mRNA vaccinations: The role of G-quadruplexes, exosomes, and MicroRNAs. Food Chem Toxicol. 2022 Jun;164:113008.
Sharma S, Fitzgerald KA, Cancro MP, et al. Nucleic Acid-Sensing Receptors: Rheostats of Autoimmunity and Autoinflammation. J Immunol. 2015 Oct 15;195(8):3507-12.
Shrotri M, Navaratnam AMD, Nguyen V, et al. Spike-antibody waning after second dose of BNT162b2 or ChAdOx1. Lancet 2021;398:385-387.
Sterlin D, Mathian A, Miyara M, Mohr A, Anna F, Claër L, et al. IgA dominates the early neutralizing antibody response to SARS-CoV-2. Science Translational Medicine. 2021;13(577):eabd2223. doi: 10.1126.
Tanji H, et al. Toll-like receptor 8 senses degradation products of single-stranded RNA. Nat Struct Mol Biol. 2015; 22:109–115.
Teijaro JR, Farber DL. COVID-19 Vaccines: Modes of Immune Activation and Future Challenges. Nat Rev Immunol (2021) 21(4):195–7.
Toro H, Fernandez I. Avian infectious bronchitis: specific lachrymal IgA level and resistance against challenge. Zentralbl Veterinarmed B. 1994 Oct;41(7-8):467-72.
Underdown BJ. IgA. In: Delves PJ, editor. Encyclopedia of Immunology (Second Edition). Oxford: Elsevier; 1998. p. 1196–9.
Vanshylla K, Di Cristanziano V, Kleipass F, et al. Kinetics and correlates of the neutralizing antibody response to SARS-CoV-2 infection in humans. Cell Host Microbe 2021;29(6):917-929.
Victora GD, Nussenzweig MC. Germinal centers. Annu. Rev. Immunol. 2012;30:429–457.
Vidarsson G, Dekkers G, Rispens T. IgG subclasses and allotypes: from structure to effector functions. Frontiers in immunology. 2014;5:520-.
Wang Z, Lorenzi JCC, Muecksch F, Finkin S, Viant C, Gaebler C, et al. Enhanced SARS-CoV-2 neutralization by dimeric IgA. Science Translational Medicine. 2021;13(577):eabf1555.
Wang Z., Muecksch F., Schaefer-Babajew D., Finkin S., Viant C., Gaebler C., Hoffmann H.-H., Barnes C.O., Cipolla M., Ramos V., et al. Naturally enhanced neutralizing breadth against SARS-CoV-2 one year after infection. Nature. 2021;595:426–431.
Yamey G, Garcia P, Hassan F, Mao W, McDade KK, Pai M, Saha S, Schellekens P, Taylor A, Udayakumar K. It is not too late to achieve global covid-19 vaccine equity. BMJ. 2022 Mar 24;376:e070650.
Ying H, Zaks TZ, Wang RF, Irvine KR, Kammula US, Marincola FM, Leitner WW, Restifo NP. Cancer therapy using a self-replicating RNA vaccine. Nat Med. 1999 Jul;5(7):823-7.
Zhang Z, et al. Structural analysis reveals that Toll-like receptor 7 is a dual receptor for guanosine and single-stranded RNA. Immunity. 2016; 45:737–748.